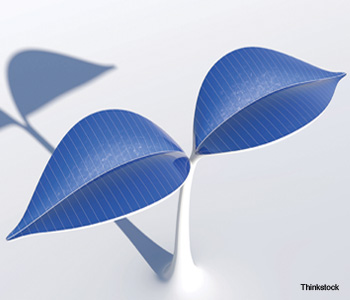
While our supply of fossil fuels is secure for now, future availability is uncertain. The demand for enough energy to sustain billions of people is rising as the global population surges, and there is only so much coal, natural gas and oil in the ground to be claimed. Whenever the world’s need for energy spikes—when fuel prices peak and shortages crop up—it becomes clear that we must develop alternative energy resources to meet our ever-increasing consumption.
Technologies that use solar photovoltaics (PV), which capture sunlight with semiconductor materials and turn it into direct electrical current, are on the rise. Solar panels are spreading across deserts and fields in the United States (as well as many other countries) and are more ubiquitous than ever atop individual homes and businesses.
However, one of the downsides to solar PV is that the energy it produces is not easy to transport. Given the outdated electrical grid in the United States, routing solar-derived power from California to Wisconsin is problematic. Delivering it to the power-hungry East Coast is an especially thorny task. What we need is a reliable way to collect and store the sun’s energy for use whenever and wherever it’s needed.
Mimicking the natural process of photosynthesis might just be the answer. Photosynthesis takes advantage of a broad spectrum of sunlight to produce and store energy inside the cellular structures of vegetation.
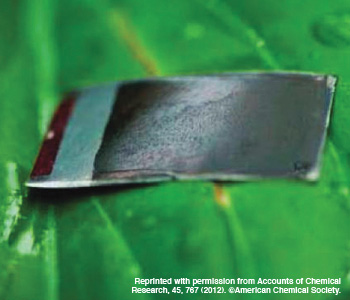
Daniel Nocera’s artificial leaf, formed of layers of nickel, cobalt and silicon. [Reprinted with permission from Accounts of Chemical Research 45, 767 (2012). ©American Chemical Society.
New fuels from sunlight and water
There are nearly a hundred physical processes involved in photosynthesis, some of which directly involve the absorption of photons and the transfer of energy via molecular “antennas” that help move electrons to their final destinations. Plants ranging from daisies to trees chiefly use chlorophyll-containing cells to effect this process (some photoactive bacteria have an analog material, dubbed bacteriochlorophyll). In chlorophyll-containing plants, the process of photosynthesis splits water molecules (H20), releasing oxygen and storing the energy produced by that chemical reaction inside a carbohydrate molecule. The energy resident in each photon is transferred into the final organic compound product, which the plant stores as adenosine triphosphate (ATP) for later use.
Using the right synthetic materials, this natural process can be used to split water molecules apart, ultimately sending the hydrogen part into fuel cells (i.e., batteries) that can easily bring the captured solar energy to where it is needed.
Artificial photosynthesis
Plants have had millions of years to improve upon photosynthesis, and most green plants are fairly efficient at it, locking down up to 6 percent of the solar energy that strikes a leaf’s surface. Modern solar PV systems can achieve three times higher efficiency. Nonetheless, artificial systems that mimic natural photosynthesis typically don’t provide direct electrical output, as does solar PV. Instead, they use readily available water sources to create a stream of hydrogen that can be stored and burned as fuel. Exploring natural photosynthesis helps tease out the secrets plants have as model systems for larger fuel production.
According to Thomas J. Meyer, Arey Distinguished Professor of Chemistry at the University of North Carolina, Chapel Hill, “The goal of artificial photosynthesis is to mimic the green plants and other photosynthetic organisms in using sunlight to make high-energy chemicals but with far higher efficiencies and simplicity of design for scale-up and large-scale production.”
Photosynthesis begins when the pigments within a plant cell act as antennas that capture photons. These antennas then generate electrons that pass the energy along to other molecules in the multistep process of energy capture, redirection and storage.
One or two types of pigments are necessary for a completely functional system: Photosystem I refers to the absorption of light via the main type of chlorophyll by itself. Photosystem II requires a second pigment.
Light-harvesting polymers must be able to absorb sunlight over a significant span of the spectrum as well, in order to not waste photons.
To compete with solar PV and other green energy systems, artificial photosynthesis research is leaning toward maximizing the efficiency of photon absorption. Generally, the way to do this is to find or develop materials that are easy and inexpensive to produce.
The road to titanium dioxide
One process that is being explored as a means of producing an affordable energy supply is the development of photocatalytic water splitting under solar light.
Decades ago, Japan’s Akira Fujishima published research on the ability of titanium dioxide (TiO2) to split water molecules into their constituent oxygen and hydrogen atoms when exposed to light. Since Fujishima’s report, interest in TiO2 has languished because it is a relatively poor absorber of visible light. Still, researchers from the Pennsylvania State University in University Park, Arizona State University in Tempe, and the University of North Texas in Denton, U.S.A., have collaborated to improve TiO2-based systems.
The team, led by Thomas Mallouk of Penn State, works with TiO2 electrodes that are sensitized to incoming light with the addition of a dye composed of ruthenium tris(bipyridine), a material known for its efficient light absorption and durability. The ruthenium dye enhances the antenna effect and can oxidize water with the help of an iridium oxide catalyst, but Mallouk and his colleagues saw that there were further efficiencies to be had by following nature a little further.
Artificial photosynthesis: A global effort
A global conference on artificial photosynthesis, called “Toward Global Artificial Photosynthesis: Energy, Nanochemistry & Governance,” was held in August 2011 on Lord Howe Island, Australia. Researchers from around the world gathered to foster international collaborations and strategies for funding through global efforts in five key areas of artificial photosynthesis:
- Global collaborations, governance and policy structures and models.
- Energy capture, including photovoltaic systems.
- Energy conversion and storage, including quantum coherence in electron transfer and hydrogen production for fuel cells.
- Carbon fixation.
- Modified and synthetic biological processes.
Experts from Australia, Finland, Germany, Japan, Sweden, the United Kingdom and the United States gave talks on artificial photosynthesis, nanotechnology and global governance.
Information about the conference, including speaker videos, abstracts and posters can be found at http://law.anu.edu.au/coast/tgap/conf.htm.
Because photosynthesis is an assembly line of electron transfer steps, the timing of each step is tightly controlled. In particular, the tyrosine-histidine mediator is essential in handing off electrons from the manganese oxide water oxidation catalyst to P680, one of the chief dye molecules in Photosystem II. In the 25 September 2012 issue of Proceedings of the National Academy of Sciences, Mallouk’s group reported that adding an artificial electron transfer mediator more than doubled the efficiency of its dye-sensitized system.
The mediator is a chemical called benzimidazole phenol (BIP). In the artificial system, BIP mediates electron transfer between the relatively fast-acting dye molecules and the slower-reacting iridium oxide. When activated by light in the blue range, the mediator-aided dye-sensitized system splits water molecules with a quantum efficiency of about 2.3 percent.
Clearing bottlenecks
“There are two major bottlenecks: light capture and charge use,” said Peidong Yang of Lawrence Berkeley National Laboratory, U.S.A., referring to the photocathode and photoanode aspects of artificial photosynthetic systems, respectively. “Utilizing the charge has been fairly inefficient at this moment.”
“There are a lot of groups jumping into the field,” he added. “But there is a lot of similarity among them.”
Using the whole spectrum of sunlight is important because gaining efficiency depends on capturing as much energy from every wavelength possible. Titanium dioxide, for example, is mainly sensitive to the blue and ultraviolet parts of the spectrum, which leaves a lot of untapped potential in the visible range.
Getting good photocurrent density is the biggest bottleneck, said Yang, who also teaches at the University of California, Berkeley, U.S.A. The cathode part of the equation is relatively set. Yang and his colleagues have explored several combinations of materials, including some based on silicon and some based on gallium phosphide (GaP). Because GaP can efficiently aid not only water-splitting but also the reduction of carbon dioxide (CO2) to other fuels, they created nanoscale wires made of GaP. They also doped the wires with zinc to optimize their charge-carrying capacity.
The group reported in the 10 October 2012 issue of Nano Letters that zinc-doped GaP nanowire systems are comparable to single crystalline semiconductor wafer devices, but easier to manipulate through alterations to the doping levels and through processing techniques. And because the synthesis is based on a low-temperature solution method, the production of such high-surface-area photoelectrode materials can be readily scaled up.
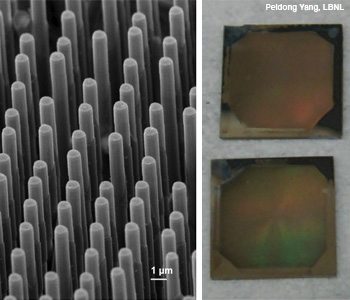
(Left) Silicon or TiO2 nanowire asymmetric arrays can act as (right) integrated photocathodes and photoanodes.
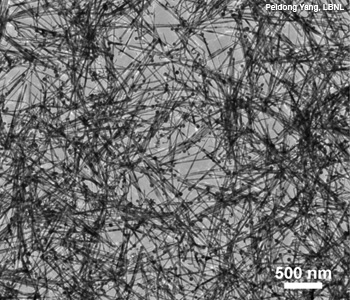
GaP nanowires are another promising material for improving the efficiency of photocathodes in water-splitting fuel generators.
On the other hand, Yang noted, the photoanode side represents a major technical barrier. Existing photoanode materials do not provide enough photovoltage or photocurrent to match the photocathode side. The Yang group is exploring semiconductor materials such as indium gallium nitride (InGaN) as a promising photoanode material. In particular, InGaN-based materials have tunable bandgaps from the infrared all the way into the ultraviolet—which is ideal for capturing the entire solar spectrum. However, the photocurrent that can be extracted from these new semiconductor candidates has not yet been optimized.
The next biggest bottleneck is the inefficient use of the charge carriers generated from photocathodes and photoanodes. In this regard, Yang’s team and many other research groups are developing better catalytic materials for water reduction, water oxidation and CO2 reduction.
Polymers as light harvesters
Metal oxides aren’t the only candidates for photon antennas. Synthetic polymers can also act in that capacity. At Melbourne University in Australia, a team led by Kenneth Ghiggino is working with materials based on the semiconducting polymers derived from phenylene vinylene.
“Synthetic polymers provide a means to mimic the light-harvesting pigment arrays that are present in natural systems such as green plants and photosynthetic bacteria,” Ghiggino said. “They are composed of many chemical structural units that are linked together. The distance between each repeating unit is quite small [a few angstroms]. If the repeat units of the polymer contain light absorbing groups [chromophores], then light absorbed by any one unit can be transported to the other units in much the same way the natural pigment arrays operate.”
By controlling the structure and shape, or conformation, of a polymer, Ghiggino added, the light-collecting ability of an artificial system can be maximized, including by developing an energy gradient to direct and trap light energy most efficiently as it is absorbed into the system.
In nature, a photosynthetic reaction center makes possible the conversion of absorbed light energy into charges that can be used to drive chemical and biological processes, such as the formation of ATP for energy storage. Precise arrangement of the antenna-like pigments in the reaction center enables charges to be separated quickly for ready transport, but slows the recombination of the separated charges so they have time to initiate the various processes required of them.
“While (the reaction center) operates remarkably efficiently,” Ghiggino said, “the arrangement is not optimized for producing other useful chemical fuels, such as hydrogen, and there is some overall energy loss. Artificial photosynthetic systems can, in principle, be designed to convert absorbed solar energy into a variety of useful fuels.”
Ghiggino and his colleagues have focused their attention on derivatives of poly[2-methoxy-5-(2’-ethylhexyloxy)-1, 4-phenylene vinylene], or MEH-PPV. The alkyl-oxygen portions incorporated into this polymer help its overall solubility and ease its processing, which is important for a prospect’s manipulability and cost-effectiveness.
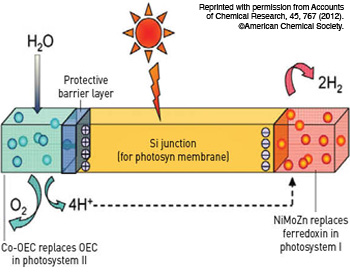
The structure of an artificial leaf produced by researcher Daniel Nocera’s team features a silicon junction that captures photons. The cobalt-based oxygen evolving complex (Co-OEC) and a nickel, molybdenum and zinc alloy (NiMoZn) perform the water-splitting function and transport the captured energy into storage.
As for the ability to manipulate a polymer’s shape, Ghiggino said, polymer chains are affected by the micro-environment in which they are situated, and this, in turn, affects the overall light harvesting process. Regulating this environment requires control of the polymer structure, film-forming conditions and subsequent thermal treatments.
Semiconductors at work
Organic polymers are not the only possible solution. Using rare-earth and other metals, researchers are extracting useful amounts of energy from sunlight.
Last year at the Massachusetts Institute of Technology, Mass., U.S.A., Daniel Nocera and his colleagues produced an artificial leaf made mostly of silicon, nickel and cobalt. The leaf is the size of a playing card, and it is about ten times more efficient than a natural leaf and can operate continuously for about 45 hours.
When the layered leaf is exposed to light and water simultaneously, a silicon junction captures incoming photons and energizes the cobalt-based oxygen evolving complex at one end and a nickel, molybdenum and zinc alloy at the other. Together, they split the water touching the device and produce hydrogen that can be put into storage. Oxygen is released from the cobalt side of the leaf, and hydrogen gas from the nickel-molybdenum-zinc side. Separating the two sides while the gases are produced would produce ample hydrogen for fuel cells.
Previously, platinum was the catalyst of choice for creating the electrolytic effect, but the nickel-molybdenum-zinc alloy performs more than adequately while being much less expensive.
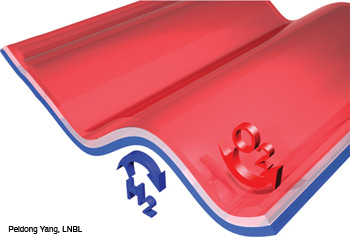
High density nanowire arrays can be used as effective photocathodes and photoanodes for solar water splitting.
The next stage
What does all this research mean to the future of artificial sun-driven fuel generation? The technology certainly isn’t ready for commercialization yet, even though test devices are being developed. Researchers like Nocera have started companies based on their successes, but production is not likely to start soon.
Yang noted that commercialization of artificial photosynthetic products can’t begin in earnest until the bottlenecks of light capture and charge usage are cleared.
Artificial photosynthesis has yet to fulfill its promise to provide a commercially viable alternative to traditional fuels, Ghiggino said, though much has been learned about the operation of the natural photosynthetic system.
Various labs can produce hydrogen and other useful fuels by mimicking the basic processes of photosynthesis, Ghiggino added, but to date all have deficiencies that prevent them from being economically or practically viable. He sees increasing the scale of laboratory systems, retaining efficiency, and developing inexpensive new materials and catalysts as the main hurdles to overcome.
“Any application that requires the efficient collection and transport of light energy to a specific site can potentially utilize light-harvesting polymers,” Ghiggino said. There is considerable interest in developing self-assembled molecular systems for solar harvesting, which would eliminate the need for extensive chemistry and thus improve manufacturability.
Some aspects of light harvesting, particularly the large-scale production of flexible organic solar cells using printing technologies, are receiving substantial commercial interest. Ghiggino contends that a 10-year timeframe for broad commercialization is realistic. However, as Nocera wrote in his seminal 2012 paper in Accounts of Chemical Research, “the artificial leaf … provides a first step down a path aligned with the low-cost systems engineering and manufacturing that is required for inexpensive solar-to-fuels systems.” Like any technology, artificial photosynthesis must bud before it blooms.
Lynn Savage is a freelance writer who specializes in science and technology. He is the owner of Savage Editorial Services, Northampton, Mass., U.S.A.